Sandalwood tree (Santalum album L., family Santalaceae), a valuable commercial tree is well-known for its heartwood fragrance. Sandalwood is a semi-root parasitic tropical tree indigenous to Southern India and Southeast Asia. The oil extracted from its heartwood is widely used in cosmetics, aromatherapy and pharmaceuticals (Srinivasan et al., 1992; Coppen, 1995). The sandalwood trees are threatened species and are mostly grown under the government regulation in India, Pakistan and Nepal. The demand for sandalwood and oil is not as much due to slow growing nature of heartwood, poor in vitro regeneration, illegal cutting down and diseases. |
The plant pathogens such as Phytophthora sp. and Fusarium oxysporum cause seedling diseases. Ascochytasantali, Macrophomina phaseoli, Asterina congesta and Sphaceloma santali causes leaf spot diseases. Ganoderma applanatum and G. lucidum cause basal stem rot disease. Among the various other diseases, sandal spike disease (SSD) caused by phytoplasma is a major disease of Santalum sp. (Mukerji and Bhasin, 1986; Ghosh et al., 1992; Srinivasan et al., 1992). The SSD occurs at any developmental stage of the tree. As the disease progresses, the new leaves become smaller, narrower or more pointed and fewer in number with each successive year until the new shoots give an appearance of fine spike. At the advanced stage, inter nodal distance on twigs becomes small, haustorial connection between the host and sandal breaks. Eventually, the affected tree dies within 2-3 years after the onset of spike disease. SSD is also known to occur in many alternate hosts such as Vinca rosea (Dijkstra and Lee, 1972; Hiruki and Dijkstra, 1973), Santalum yassi, Dodonaea viscosa and Zizyphus onoplea (Hull et al., 1970). In Karnataka, the growing stock has been reduced by 25% due to SSD compared to last two decades (Swaminathan et al., 1998). |
SSD was reported to be caused by Candidatus Phytoplasma asteris strain belonging to subgroup 16SrI-B (Kirdat et al., 2019). The genus, phytoplasma are intracellular plant pathogens derived from gram positive a bacterium belongs to the class Mollicutes. Phytoplasmas are pathogenic parasites of plant and insects. Phytoplasmas are non-culturable, phloem restricted prokaryote that lack typical cell wall and are resistant to many antibiotics. Transmission of phytoplasma mainly occurs through phloem feeding insect vectors and infected plant materials. In general, phytoplasmas cause different types of symptoms such as stunting, phyllody, witches’ broom, yellowing resulting in serious loss of yield in many economically important agricultural and horticultural crops worldwide (Arunkumar and Joshi, 2012). |
Sandalwood spike disease was first reported in Indian sandal (S. album) in 1899 from Coorg state of Southern India (McCarthy, 1900; Barber, 1903). Over a million SSD infected trees have been removed in Mysore and Coorg states between 1903-1916 (Da Silva et al., 2016). Researchers had different opinions on the cause of SSD such as imbalanced circulation of sap (Hole, 1917; Venkatarama, 1918) and virus or virus like microorganism (Coleman, 1923; Dijkstra, 1968). In 1969, the presence of mycoplasma like bodies was confirmed in phloem cells of SSD infected trees through electron microscopy (Dijkstra and Le., 1969; Hull et al., 1969, Varma et al., 1969). SSD induces characteristic phytoplasma symptoms such as chlorosis, shortened internodes, reduced leaf size with a bushy appearance and stem show a spike like appearance (Barber, 1903; Hole, 1917). Infected young trees generally die within a year or two after the appearance of spike symptoms (Swaminathan et al., 1998; Balasundaran and Muralidharan, 2004). The production of S. album is significantly affected by SSD in Kerala and Karnataka (Swaminathan et al., 1998; Kumar, 2014; Arunkumar et al., 2016). The incidence of SSD in Karnataka and other regions in Southern India has increased since 1990 (Kumar, 2014). Survey among 24 Sandal plantations from five districts in southern Karnataka for assessment of SSD incidence revealed the occurrence of spike disease ranging from 0-18 per cent. The maximum incidence (18%) was recorded in Sirataluk of Tumkur district, followed by Shimoga (1.65%). The least incidence (0.33%) was recorded in plantations of Tygarthi village (Murali et al., 2019). |
Even though the disease was reported a century ago from India, very few researches have been undertaken to understand the molecular nature of infection and management of pathogen. Diagnosis of SSD is very crucial for authentic identification. Phytoplasmas are intracellular parasite found in sterol rich phloem tissues of plant or in haemolymph of insect vectors. The detection of phytoplasma is challenging due to low titre, seasonal fluctuation and uneven distribution in planta, particularly in woody crops (Garcia-Chapa et al., 2003). The latency period of phytoplasma vary from several months to years before symptom appearance makes it more difficult for early detection and diagnosis. The distribution of phytoplasma in entire plant system is again a time taking process as a result there is a less chance of detection in the asymptomatic branches. The inhibitory enzymes, polysaccharide and polyphenolic compounds present in woody plants interfere with phytoplasma DNA isolation and further nucleic acidbased detection. Various approaches from biological to molecular techniques are employed for the detection of phytoplasma. In this chapter, we summarise the work conducted in the area of diagnosis of SSD. |
Unique molecular features of phytoplasma Phytoplasma genome consists of single chromosome with an average size of 600 - 880 kb and extra chromosomal plasmids. The genome contains the basic cellular functional genes responsible for replication, transcription, translation and protein translocation (Jung et al., 2003). Phytoplasma doesn’t contain gene involved in several biosynthetic pathways, instead they completely depend on the host cell machinery. Mycoplasmas lack genes involved in tricarboxylic acid cycle and biosynthesis of sterol, fatty acid, de novo nucleotide and most amino acids (Razin et al., 1998). Phytoplasma have also lost many metabolic genes in comparison to mycoplasma (Oshima et al., 2004). The unique feature of phytoplasma that differ from mycoplasma and other bacteria is the absence of the gene encoding F1F0-type ATP synthase, i.e., involved in synthesis and hydrolysis of ATP. The two secretion systems found in phytoplasma are Sec system and YidC system. The Sec system essential for cell viability is involved in integration and secretion of proteins into host cell cytoplasm. The YidC system is involved in integration of membrane proteins (Oshima et al., 2013). The unique phytoplasma virulence factor TENGU causes sterility in plants by down regulating the jasmonic acid and auxin pathways. Secreted proteins and virulence factors of phytoplasma play a major role in symptom expression and survivability of the primitive bacteria inside the host cell. The fine diagnosis of phytoplasma is based on the specific membrane protein and genome sequence. The ribosomal RNA (16S and 23S) genes were used as a basis of molecular detection and classification of phytoplasma. Biological diagnosis Diagnostic symptoms Biological method was the basis of identification of phytoplasma disease in earlier days, which included symptomology, host range, collateral hosts and transmission. Symptomatology was the major criterion employed in diagnosis of the disease caused by phytoplasma (Lee and Davis, 1992). SSD shows prominent yellowing of leaves with witches’ broom appearance in infected trees. Sandal trees of all stages are affected by the phytoplasma and the trees die prematurely with the onset of spike disease. SSD shows two types of spike symptoms such as rosette spike and pendulous spike. The rosette spike is more commonly found in infected trees. The symptoms appear in one part of tree and gradually it spreads throughout the tree. Young leaves become smaller, chlorotic, sometimes with purplish tinges and branches tend to stand out stiff with upright growth exhibiting a spike like appearance. Subsequently, the affected branch becomes bushy with distinct yellowing of leaves, which turn reddish shortly before the death of the tree (Fig. 1). The infected part of plant doesn’t flower or bear any fruits, while the unaffected branch of the tree continues to bear flower and fruits. The haustoria and root tips of the infected sandal tree are degenerated. Whereas, in case of pendulous spiked symptom, the haustoria and root tips do not die. The infected shoots grow apically and have a drooping effect without rosette symptoms in younger leaves. Graft and dodder transmission In addition to the diagnostic symptoms, the successful transmission of the causal agent from the disease plant to a healthy indicator host plant is an important biological criterion of identification of a pathogen. Phytoplasma being phloem borne is not sap transmissible, and therefore, graft, dodder, and insect transmission methods were attempted to transmit the SSD. Coleman (1917) described the graft transmission of SSD through infected buds, barks and twigs. The natural root grafting through haustorial connections between healthy and infected plants could contribute up to 6 to 7% of SSD transmission. Later, in 1930, Sreenivasaya reported that, the disease could also be transmitted through infected leaves by inserting it between the wood and bark tissues of healthy plant. Dodder mediated transmission of SSD to Vinca rosea was reported by Nayar and Ananthapadmanabha (1970). The infected V. rosea plants showed vein clearing and interveinal chlorosis symptoms. In 1972, Dijkstra and Lee demonstrated that the SSD can be transmitted between sandal tree and V. rosea plants and vice versa. The SSD infected V. rosea plants developed witches broom symptom, while the dodder strand was connected between the V. rosea and infected sandalwood tree. The dodder also could transmit the disease from SSD infected V. rosea back to sandalwood trees. The infection was confirmed by the presence of mycoplasma-like bodies in the test plant tissues. Indicator hosts Collateral hosts play a major role in pathogen survivability in absence of the main host. Lantana camera and Pongamia glabra are reported as symptomless carriers of SSD (Nayarand Srimathi, 1968; Kristensen, 1960). Phytoplasma disease like symptoms was observed in many plants such as Zizyphus oenoplea, Vinca rosea, Dodonea viscosa, Eucalyptus grandis, Stachytarpheta jamaicensis, Dendrocalamus strictus etc., grown near the sandalwood tree. The report also indicated that from the collateral hosts viz., Zizyphus sp. and sesamum with spike symptoms; the disease might get transmitted to sandal trees within a period of 3-5 years. The causal agent could also be transmitted between the collateral host and sandal trees and vice versa (Nayar, 1981). Vinca rosea has been shown as an excellent experimental indicator plants for SSD or phytoplasma in general. Vector transmission As insect vector transmits virus or virus like pathogens with an extraordinary biological specificity, the vector transmission by a specific insect vector was considered as the convincing identification evidence of these types of pathogens in the absence of biochemical or molecular diagnostic assays in the early phase of the development of the subject. The first evidence of insect vector transmission of phytoplasma was demonstrated with a leafhopper, Macrosteles fascifrons that transmitted infectious agent from aster yellows (Kunkel, 1926). The insect vector transmission of phytoplasma was demonstrated way before the discovery of phytoplasma (Doi et al., 1967). Several leafhopper, planthopper and psyllid have been identified as the vectors of phytoplasma. Dover (1932) suspected that SSD can be transmitted by insects. Later in 1940, Rangaswami and Griffith reported that insect vectors are primary source of transmission of SSD in nature. Insects such as Moonia albimaculata Dist., (Dover and Appanna, 1933), Coelidia indica Walker (Rangaswami and Griffith, 1940) and Nephotettix virescens (Shivaramakrishnan and Sen-Sarma, 1978) were found to be vectors of SSD. Some of the vectors like M. albimaculata and C. indica need further confirmation (Sen-Sarma, 1982). The successful transmission of SSD through the known vector species is an important confirmatory bioassay of SSD. The authenticity of some of the vectors like M. maculata and C. indica needs further confirmation (Sen-Sarma, 1982). The successful transmission of SSD through the known vector species is an important confirmatory bioassay of SSD. It appears that there could be multiple vectors of SSD phytoplasma. However, after 1980s, there is a lack of vector transmission studies of SSD and as a result of that the data pertaining to the transmission parameters, efficiency of transmission by the different vectors listed in the literature are lacking. Microscopic diagnosis Light microscopy Light microscopy is used as a preliminary and indirect method to detect phytoplasma. Light microscopy is limited when the pathogen titre is low in the disease tissues. Detection of abnormal levels of wounded callose produced in response to injured phloem is an indirect method for diagnosis of phytoplasma. The routinely used dyes for detection of phytoplasma are Diene’s stain, which produce blue colour (Fig. 2) and DAPI (4’-6-diamidino-2-phenylindole), a DNA binding fluorochrome (Dienes et al., 1948; Seemuller, 1976). Ananthapadmanabha et al. (1973) used Glemsa and Dienes’ stain for the detection of SSD phytoplasma. Hoechst 33258, a DNA binding fluorochrome was used to detect the SSD phytoplasma and found that the fluorescence intensity was higher in stem and petiole compared to root tissues. These studies conclude that fluorescent microscopy is more sensitive than light microscopy for detection of SSD phytoplasma (Ghosh et al., 1985, Rangaswamy, 1995). Electron microscopy Transmission Electron Microscopic (TEM) studies led to the discovery of phytoplasma, a new class of pathogen causing plant diseases, which were previously suspected to be caused by viruses. Phytoplasma was first observed in the ultra-thin sections of samples from different plant diseases such as yellows disease of aster, witches’ broom of potato, paulownia, and mulberry dwarf, which were referred at that time as mycoplasma-like organisms (MLO) (Doi et al., 1967; Maramorosch, 2011). Phytoplasmas resemble animal or human mycoplasmas morphologically and share several characteristics. In 1994, the MLO associated with plant was referred as phytoplasma, (Sears and Kirkpatrick, 1994). The structural characteristics of phytoplasma can be studied only by electron microscopy (Marcone and Ragozzino, 1996). The phytoplasma characteristics such as pleomorphic nature, unicellular, cells delimited by thin membrane and absence of cell wall can be observed by TEM. TEM is routinely used as a confirmatory technique to identify many phytoplasma since 1967. In the early stage of study, SSD was thought to be caused due to the physiological disorder of the roots caused by the imbalance of sap movement within the plant (Hole, 1917, Venkatarama, 1918) or suspected to be caused by fungi (Latham, 1918), ultramicroscopic bacteria (Fischer, 1918) or virus (Coleman, 1917, 1923). Later, in 1969, mycoplasma like bodies was found in sandal spike phloem tissues by three different groups using TEM (Dijkstra and Le, 1969; Hull et al., 1969; Varma et al., 1969). Therefore, the usage of TEM disproved the association of other pathogen concepts and confirmed that SSD is caused by a phytoplasma. Scanning electron microscope (SEM) uses a beam of focussed electrons to scan the sample surface and records the interaction between electron and sample to create a three-dimensional magnified image. SEM offers three dimensional topographical forms of phytoplasma structure with reduced resolution compared to twodimensional, higher resolution TEM. SEM permits rapid examinations of large areas of plant tissues, whereas TEM can be employed to view at the cellular level (Marcone and Ragozzino, 1996).SEM was introduced for studying mollicutes by Biberfeld and Biberfeld (1970) and subsequently used to observe many phytoplasmas (Bove, 1984). Thomas and Balasundaran, 2001, observed the purified SSD phytoplasma using SEM. The phytoplasma showed spherical/elliptical structure with an average size of 1.0 mm diameter in phloem tissues of diseased tissue. The significant feature of mollicute, the attachment of phytoplasma to inner surface of phloem tissues was also observed using SEM. Protein based methods Immuno-assays techniques are highly specific for the identification and quantitation of pathogen. Phytoplasma was purified using differential centrifugation (Clark et al., 1983; 1989), Celite pad filtration (Sinha and Benhamou, 1983) and percoll density gradient centrifugation (Jiang and Chen, 1987) techniques were used to prepare antigen to raise polyclonal antisera. Production of high titre antisera requires extremely purified antigen. The efficiency of purified antigen is reduced due to several rounds of centrifugation. In 1975, Nayar and Ananthapadmanabha used ammonium sulphate purification method to purify SSD phytoplasma. The polyclonal antiserum was produced in rabbit using the purified SSD phytoplasma antigen. Gel diffusion and agglutination tests were employed to detect the phytoplasma with a low titre (1:250) antiserum. The antiserum successfully detected the phytoplasma in SSD infected sandal and Catharanthus roseus. Later, the SSD phytoplasma purified by the differential centrifugation method was used to raise a better quality antiserum (Rangaswamy, 1995). The antiserum was tested by indirect ELISA to detect the SSD phytoplasma from the infected sandal and Catharanthus roseus at 1:1000 dilution. The antibody could detect the phytoplasma in infected sap at a dilution of 1:200. Thomas and Balasundaran (2001) used differential filtration technique for the purification of phytoplasma in order to produce high quality antigen. The antisera detected the SSD phytoplasma at a titre of 1: 2000. Nucleic acid-based method Polymerase chain reaction (PCR) is the most versatile tool for detecting phytoplasmas in plant and insect hosts (Smart, 1996). Nested PCR followed by restriction fragment length Polymorphism (RFLP) is routinely employed for detection and identification of phytoplasma (Saeed and Cousin, 1995). The molecular techniques are more sensitive, specific and reliable for routine detection, quantification and differentiation of phytoplasma at very low titer compared to immunological and microscopic methods. PCR followed by sequencing formed the basis for classification and identification of phytoplasma and strain differentiation. Top RFLP RFLP has been extensively used as a tool for identification and classification of phytoplasma since 1990s. In this method, a set of 17 restriction enzymes are used to generate a banding pattern over 1.25-kb DNA segment amplified through nested PCR from the 16S rRNA sequence (Lee et al., 1998). The new group and subgroup are assigned when the similarity coefficient of the polymorphic banding pattern is less than 0.85 and 0.97, respectively with that of previously established members (Zhao and Davis, 2016). The 16S rRNA based RFLP was further improved with an easy-to-use webbased tool iPhyClassifier, where 16SrDNA sequence (Fig. 3) is used to generate a computer simulated RFLP pattern (Zhao et al., 2009). This method is convenient to use as RFLP pattern similarity coefficients and overall sequence similarity scores instantly suggest the group and/or subgroup position of the candidate to be identified. |
Initially, restriction analysis of 16S rRNA PCR product with AluI restriction enzyme differentiated phytoplasmas into four groups, with SSD being classified in the first group (Ahrens and Seemuller, 1992). Seemuller et al. (1998) classified phytoplasmas into 20 groups and placed sandal spike phytoplasma in the Aster yellows group (Group I), and unclear subgroup. The later study with RFLP analysis of 16S rRNA nested PCR products and the sequence identity showed that the SSD phytoplasma is closely related to aster yellows subgroup 16SrI-B (Khan et al., 2008). The restriction pattern of the virtual RFLP gel of the available sequence of SSD phytoplasma 16S rRNA gene using iPhyClassifier confirmed the identity of 16SrI-Bsubgroup. One isolate, SW03 (MK627527) SSD phytoplasma isolate, however showed different virtual restriction banding pattern compared to the other SSD phytoplasma isolates (Fig. 4). |
Top PCR Phytoplasma is routinely detected by PCR (Bertaccini et al., 2019). Several primers were developed based on the sequence in the 16S rRNA, internal transcribed spacer and 23S rRNA region for the detection of different phytoplasma belonging to the ribosomal groups and subgroups. The most commonly used PCR method is nested PCR, where two rounds of PCR are performed with the two pairs of universal primers. The first PCR is performed on the total plant DNA isolated from the phytoplasma infected plant with the forward primer P1on16SrRNA: aagagtttgatcctggctcaggatt and the reverse primer P7on23SrRNA: cgtccttcatcggctctt, which generates 1.8 kb amplicon. In the second PCR, the product of first PCR is used as template with the nested primers on 16SrRNA, forward primer R16mF2: catgcaagtcgaacga and reverse primer R16mR1cttaaccccaatcatcgac, which generates 1.2 kb amplicon (Deng and Hiruki, 1991; Gundersen and Lee, 1996). The first PCR based detection of SSD phytoplasma was reported in 1999 by Thomas and Balasundaran, where they were successful in amplification of 558 bp amplicon from SSD affected leaf samples by using Taq DNA polymerase as well as Dynazyme II polymerase. Khan et al. 2004 found that the direct PCR or southern hybridization in the total DNA isolated from the infected sandal leaves showed neither any positive amplification nor any signal with the specific probes. The reason of the failure of detection was attributed to the low phytoplasma concentration in the total DNA samples. However, Khan et al. 2004 developed a sensitive nested PCR using the above universal primers (P1 & P7 and R16mF2&R16mR), which could efficiently detect the SSD phytoplasma in the highly diluted (60,000 times) first PCR product. The nested PCR is highly sensitive and reliable diagnostic method for the screening of sandal trees and insect vector. |
Real time PCR Phytoplasma occur in low concentration and subjected to seasonal titre variation and uneven distribution in the different parts of plant tissues. Although, nested PCR is a convenient diagnostic method for the routine usage, it is not suitable for quantification of phytoplasma load in plant tissues. Real time PCR (qPCR) is a versatile quantitative PCR technique, which utilises TaqMAN probe specific to the 16S rRNA gene for the detection of phytoplasma with higher sensitivity and specificity. The 16S rRNA qPCR is ten times more sensitive than the conventional nested PCR (Christensen et al., 2004). Universal and group specific qPCR primers and probes targeting 16S rRNA have been established for several phytoplasmas (Galetto and Marzachi, 2010). However, the usefulness of qPCR is yet to be exploited for the SSD phytoplasma studies. Loop-mediated isothermal amplification (LAMP) The PCR targeting 16S ribosomal genes is the principal molecular technique for the detection of phytoplasma. PCR can be easily performed, but it requires a well-equipped laboratory. LAMP was invented for the isothermal amplification of DNA during 2000 (Notomi et al., 2000). In this method, Bst DNA polymerase and a set of four primers that recognise six distinct sequences on the target DNA are key reagents. The Bst DNA polymerase amplifies the target DNA at 60 to 65°C by 30 to 60 min. The amplified targets in LAMP reaction can visualize through a colorimetric assay by using hydroxyl napthol blue that detect the magnesium pyrophosphate by-product in successful LAMP amplification (Goto et al., 2009).LAMP is an easy, rapid, and sensitive amplification technique, which can be performed for the diagnosis of phytoplasma diseases in laboratories that lack the advanced facilities (Bekele et al., 2011; Obura et al., 2011; Sugawara et al., 2012). LAMP assay has been used to detect the aster yellows group phytoplasma in different crops (Tomlinsona et al., 2010), but so far it has not been utilized for the detection SSD phytoplasma. Development and evaluation of a one hour DNA extraction and loop-mediated isothermal amplification assay for rapid detection of phytoplasmas. Genome sequence-based identification The molecular techniques are more sensitive, specific and reliable for routine detection, quantification and differentiation of phytoplasma at very low titer compared to immunological and microscopic methods. Sequencing of 16S ribosomal gene followed by RFLP formed the basis for identification and classification of phytoplasma. However, a single gene-based classification is sometimes unreliable and adoption of multilocus gene sequence is suggested for better classification of phytoplasma (Glaeser and Kampfer, 2015). Several protein coded genes have been proposed as molecular markers for the classification of phytoplasma, for example, rpl22 and rps3 (Lim and Sears, 1992, Martini et al., 2007), preprote intranslocase SecY (Lee et al., 2006), chaperonin 60 (groEL/cpn60) (Mitrovic et al., 2011, Dumonceaux et al., 2014), elongation factor Tu (Schneider and Gibb, 1997), replication initiation protein DnaD, DegV family protein, TIGR00282 family metallo phospho esterase, preprotein translocase SecY and RluA family pseudouridine synthase (Cho et al., 2020). Identification and classification of SSD phytoplasma is limited by the lack of adequate genome sequence resource. So far, only 16 sequences of SSD phytoplasma from 16S rRNA gene have been submitted in NCBI database (Table 1). Out of 16 sequences, only 11 sequences cover the 1.2 kb region of 16S rRNA gene. The evolutionary analysis of 1.2 kb gene of SSD phytoplasma and other known phytoplasma sequences available at USDA phytoplasma classification database showed that the SSD phytoplasma isolates are found closely related to Ca. P. asteris and Ca. P. lycopersici and they are clustered in a single clade except one isolate, SW03 (MK627527), which is found to be closely related to monocot infecting phytoplasma, Cynodon dactylon and Oryza sativa (?) (Fig. 5). The SSD phytoplasma and other known phytoplasma 16S rRNA gene pair wise similarity scores were obtained and the isolates were clustered by rooted neighbour joining phylogenetic tree to get the colour coded matrix by species demarcation tool. The nucleotide sequence analysis of the 16S rDNA gene of SSD phytoplasma and other known phytoplasma groups varied between 86 to 100% nucleotide sequence identities. SW03 isolate showed close nucleotide sequence identity with monocot infecting phytoplasmas, whereas, other SSD isolates showed close sequence identity to Ca. P. asteris (Fig. 6). Top Conclusion Reliable and sensitive diagnosis is required for the studies leading to development of management options of SSD phytoplasma. Several conventional and molecular techniques have been used to study the nature of SSD phytoplasma. Biological or histopathological assays are time consuming and often not effective for precise identification. Lateral flow and LAMP assays based visual detection of SSD phytoplasma is required for the rapid and large scale screening of plant as well as vector samples in the forest and nurseries. Nucleic acidbased detection like nested PCR and qPCR are more reliable approach for the detection of SSD phytoplasma. Molecular diagnosis relies on the availability of genome sequence data. Very limited sequence resource i.e., only 16S rRNA sequence is available for SSD phytoplasma. Sequence identity and phylogeny-based identification provide the finest and measurable difference between the closely related phytoplasma isolates, which are otherwise difficult to resolve even by the molecular tests like PCR or RFLP. Recent studies on SSD phytoplasma indicate the possibility of association of diverse phytoplasma in SSD. Generation of full genome sequence resource of SSD phytoplasma isolates from different geographical regions is essential for better understanding of the pathogen and development of proper diagnosis. |
Top Figures Fig. 1.: Sandal spike disease (SSD) symptoms. A. A healthy twig showing dark green leaves and flowers; B. A SSD affected twig showing chlorotic and small leaves; C. A healthy sandal tree; D. An affected tree showing chlorotic bushy foliage; E. An affected tree with dead branches
| 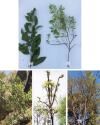 | |
| Fig. 2.: Diene’s staining of sandal stalk showing phytoplasma bodies in phloem cells
| 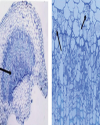 | |
| Fig. 3.: Diagrammatic representation of Aster yellows witches broom phytoplasma (NC_007716) phytoplasma rRNA operon with 16S rRNA gene, intergenic spacer and 23S rRNA gene.
| 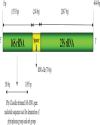 | |
| Fig. 4.: Virtual gel restriction fragment length polymorphism patterns of 16S rDNA of sandal spike phytoplasma isolates. a. SW04 (accession no. MK627541) isolate showed 99.8% similarity with Candidatus Phytoplasma asteris (accession no. M30790) and found to be Candidatus Phytoplasma asteris related strain; b. SW03 (accession no. MK627527) isolate showed 98.0% similarity with Candidatus Phytoplasma cynodontis, GenBank accession: AJ550984 and found to be Candidatus Phytoplasma cynodontis related strain
| 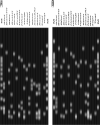 | |
| Fig. 5.: Phylogenetic analysis of phytoplasma 16S rDNA sequences belonging to different groups. The 16S rDNA sequences were trimmed using the iPhyClassifier web tool available online at usda.gov website. The sandal wood 16S rDNA sequences were underlined
| 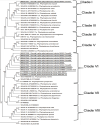 | |
| Fig. 6.: Pairwise identity of Sandal wood phytoplasma 16S rDNA gene nucleotide sequences (indicated with dotted box) with other known phytoplasma groups. 16S rDNA gene (~1200 bp) nucleotide sequences were trimmed using iPhyClassifier web tool available at usda.gov. The matrix was generated using sequence demarcation tool version 1.2.
| 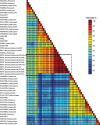 | |
|
Table Table 1.: Sandal spike disease phytoplasma sequence of 16S ribosomal RNA submitted in NCBI GenBank database.
| Sl. No. | Accession | No of Bases | Location | Author | 1 | MK627527 | 1248 | Marayoor, Kerala | Kirdat et al. (2019) | 2 | MK627528 | 1246 | Marayoor, Kerala | | 3 | MK627529 | 1246 | Marayoor, Kerala | | 4 | MK627536 | 1247 | Marayoor, Kerala | | 5 | MK627537 | 1246 | Marayoor, Kerala | | 6 | MK627541 | 1246 | Marayoor, Kerala | | 7 | MK627542 | 1246 | Marayoor, Kerala | | 8 | MK627543 | 1245 | Marayoor, Kerala | | 9 | MK627544 | 1247 | Marayoor, Kerala | | 10 | EF198362 | 1427 | Karnataka | Khan et al. (2009) | 11 | EF050071 | 1427 | Karnataka | | 12 | DQ092357 | 1149 | Mysore, Karnataka | Khan et al. (2006) | 13 | DQ078260 | 590 | Mysore, Karnataka | Khan and Srivastava (2005) | 14 | DQ078261 | 542 | Mysore, Karnataka | | 15 | DQ078262 | 526 | Mysore, Karnataka | | 16 | DQ078263 | 411 | Mysore, Karnataka | |
| |
|